Simulation Analysis of High-Mass X-Ray Binaries
This project uses the COSMIC population synthesis code (Breivik et al. 2019), which is based on the BSE binary stellar evolution code (Hurley et al. 2002). BSE implements a statistical binary evolution scheme that does not explicitly calculate detailed stellar physics. COSMIC works with BSE to evolve large binary populations in a python environment. For more details on how COSMIC works, please refer to the complete COSMIC documentation.
We simulate large populations of binary black holes with COSMIC and identify merging systems that evolve through high mass x-ray binaries (HMXBs). From these populations, we can determine the frequencies of black hole mergers and non-mergers (alive systems), along with the relative occurence of HMXBs. The resulting statistics from a simulation of ~50,000 binary black holes at one-tenth solar metallicity are displayed in the table below.
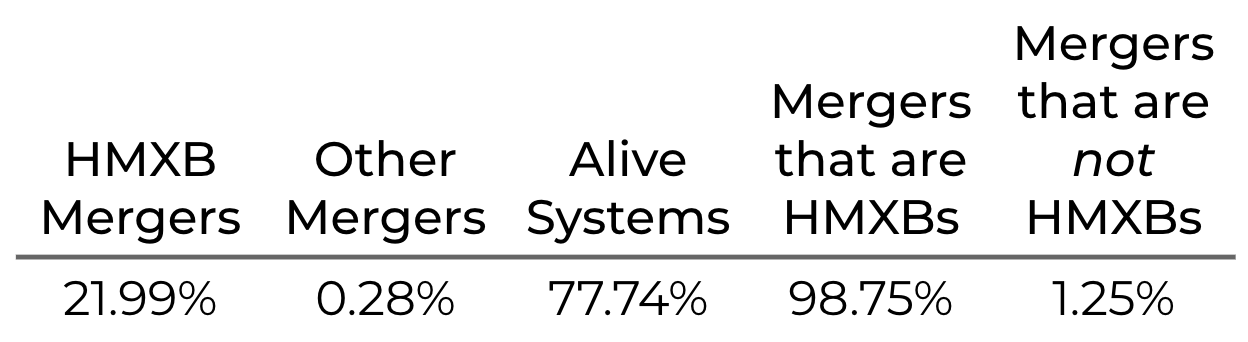
We find that most binary black hole systems are still alive, but of those that have merged, almost all have HMXB sources. The merging systems that do not originate from HMXBs come from binary stars that follow parallel evolutionary tracks. In other words, both stars in the binary start with nearly identical masses and reach stages of burning at the same time as each other; there is never a time when the objects have drastically different stellar types. From these results, we conclude that HMXBs are the most common progenitor of simulated binary black hole mergers.
Once we identify merging HMXB systems, we examine their evolutionary stages and properties. We are primarily interested in the luminosities of these systems because we want to know if they are observable. These systems emit light when the black hole accretes matter from its companion star; the resulting luminosity is proportional to this mass accretion rate. The lower limit of observable luminosities is between 1030 and 1035 ergs per second. We calculate the black hole accretion luminosity according to Marchant et al. 2017. As a first analysis step, we take the peak HMXB luminosity to be that at the maximum black hole mass accretion rate. Results from this calculation for ~550 HMXBs are displayed in the histogram below.
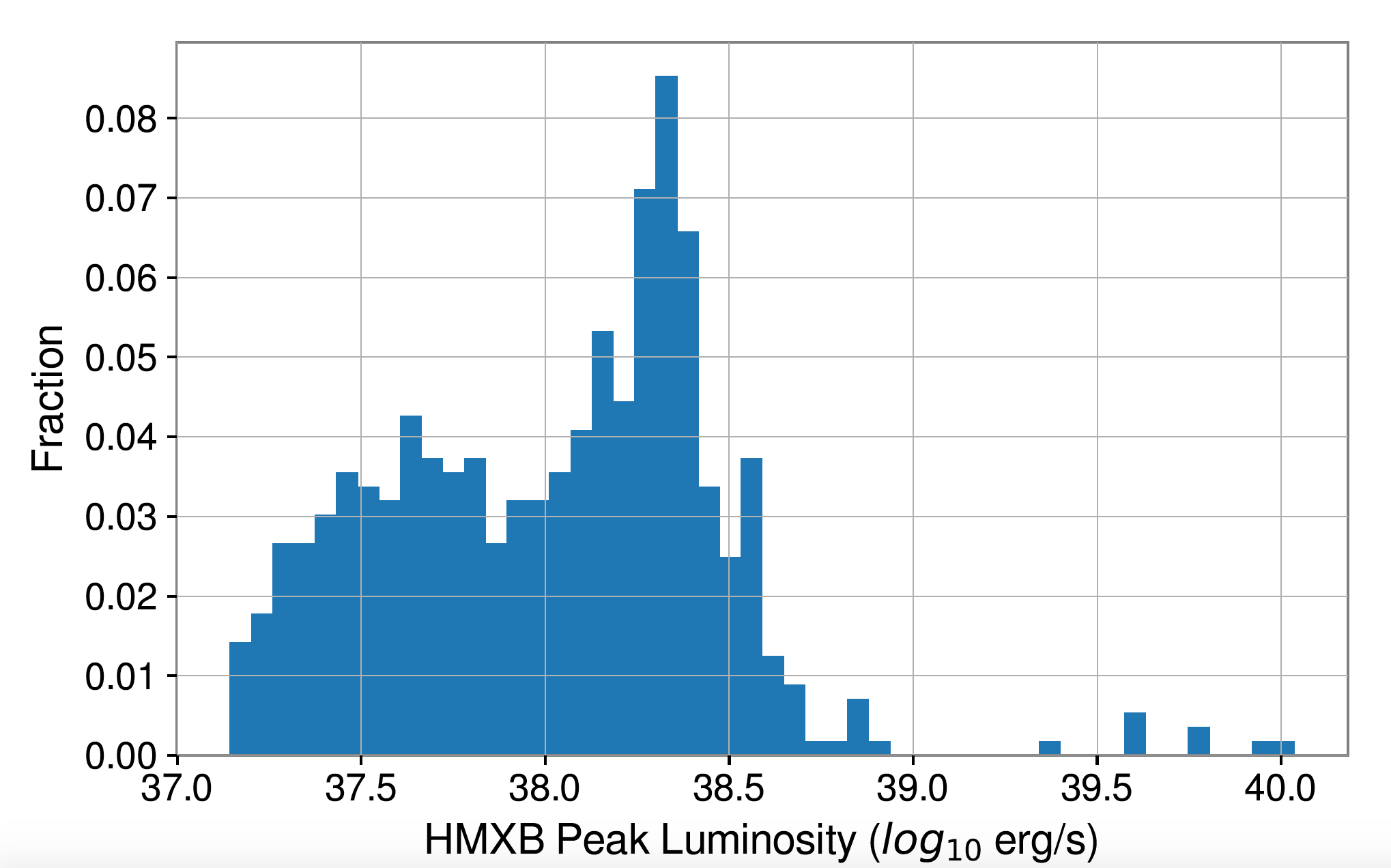
We find that all simulated HMXBs reach peak luminosities above the observable x-ray minimum. This implies that the majority of LIGO sources for BBHs should be x-ray observable at some point in their evolution. One crucial factor we have not yet considered is the time span of this observable emission. If HMXB luminosities are bright but only for a short period of time, the probability we will see them is small. However, if the emission time is non-negligable relative to the Hubble time, the probability we will see them is significant. This is the next upcoming step in our analysis.
We also determine if our simulated HMXBs can be observed by LIGO. We identify the final merging black hole masses for systems that evolve from HMXB sources and weight these systems according to LIGO's sensitivity scaling presented in Fishbach & Holz 2017. This weighting is proportional to the (primary mass + secondary mass)2.2. The resulting 2D histogram of masses with weighted points is displayed below.
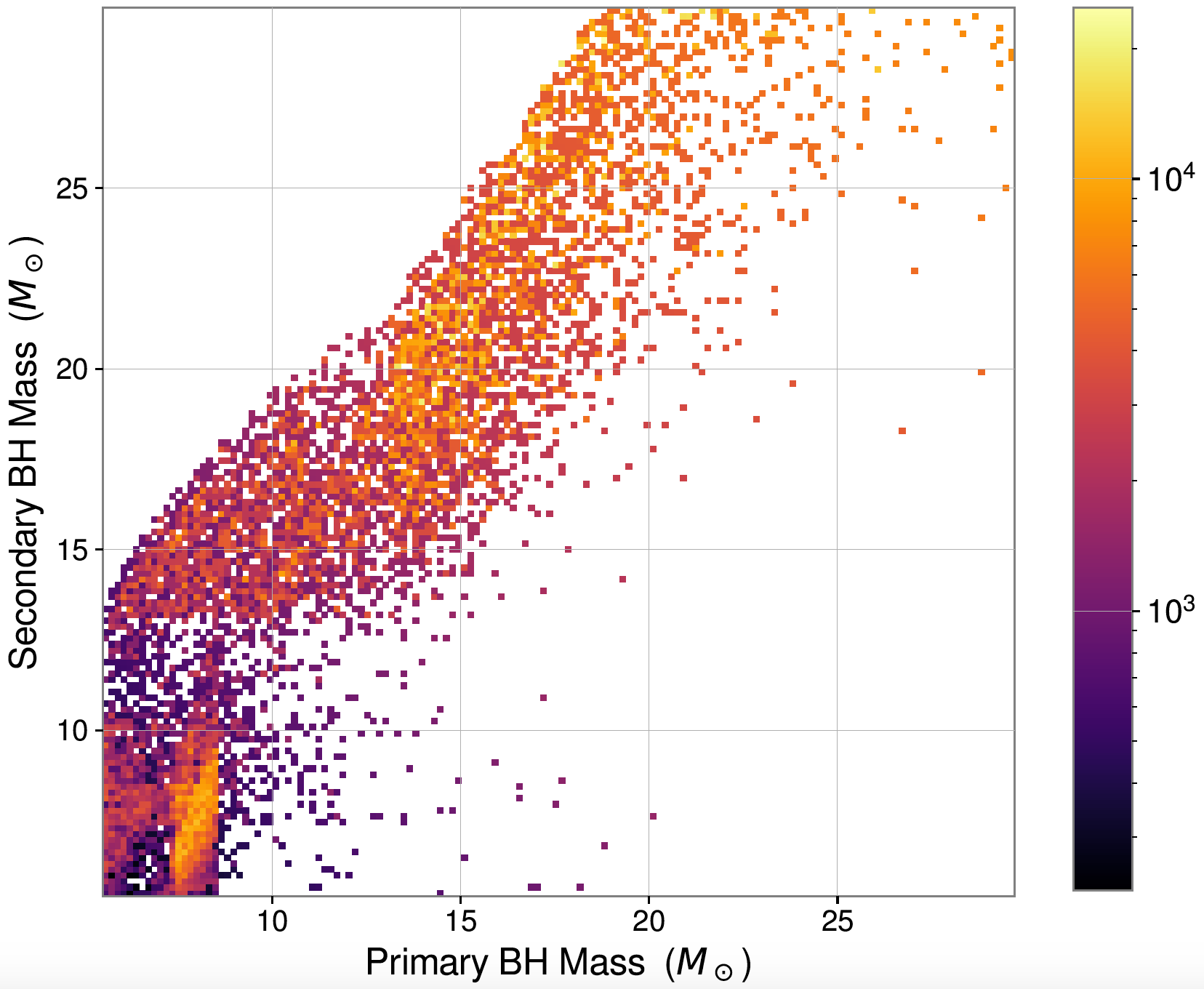
From this histogram, we see that LIGO should be able to detect lower-mass systems that are very populous and higher-mass systems that are less populous. In either case, LIGO can theoretically detect simulated merging black holes that evolve from HMXB progenitors. However, there are aspects of these results that we do not understand, primarily the fact that the secondary black holes are consistently more massive than the primaries. In a first attempt to understand this behavior, we identify the major evolutionary stages of these systems and attempt to find patterns in the evolution that could lead to this outcome. We find that in many cases, significant mass transfer happens from the primary to the secondary when the primary is still a main sequence star; its mass is drastically reduced early-on in the evolution. Later in the evolution, these same systems do not enter a second common envelope phase, which is the mechanism traditionally thought to heavily influence the final mass ratio of the system. We re-create the above histogram with points sorted according to the presence or absence of a second common envelope in the binary evolution. These results are displyed below.
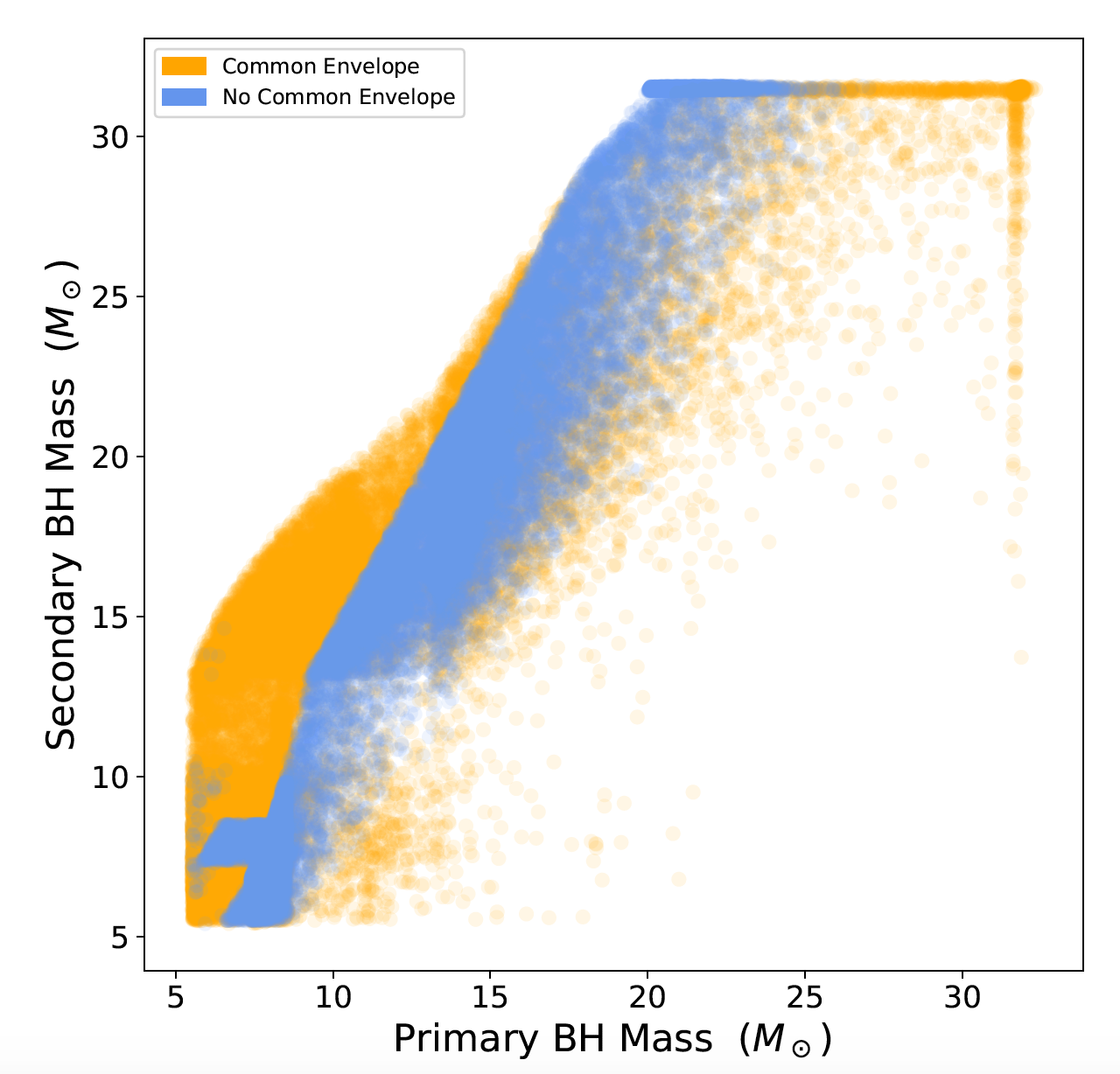
We find that 56% of all simulated HMXB black hole mergers undergo a second common envelope phase during evolution. However, while there are clear patterns and structures in the final masses relative to the second common envelope phase, we are not sure what the exact nature of this relationship is. Future project steps will include a more detailed analysis of binary evolutionary tracks and corresponding parameters at specific stages of evolution.
The final portion of this project compares BSE evolutionary tracks of single binary systems with simulations using the MESA stellar evolution code (Paxton et al. 2011). We are interested in this comparison because we want to identify differences between BSE and MESA, primarily because MESA solves equations of stellar structure for binaries at each time step and explicitly implements more rigorous physics than BSE. In other words, we want to examine the accuracy of BSE relative to MESA in order to put contraints on our COSMIC results. We input the same initial stellar masses, orbital periods, and eccentricties to BSE and MESA and confirm that both simulations result in binary black hole mergers. We then calculate black hole accretion luminosities for both systems and compare their evolution over time. An example of such a comparison is displayed in the plot below.
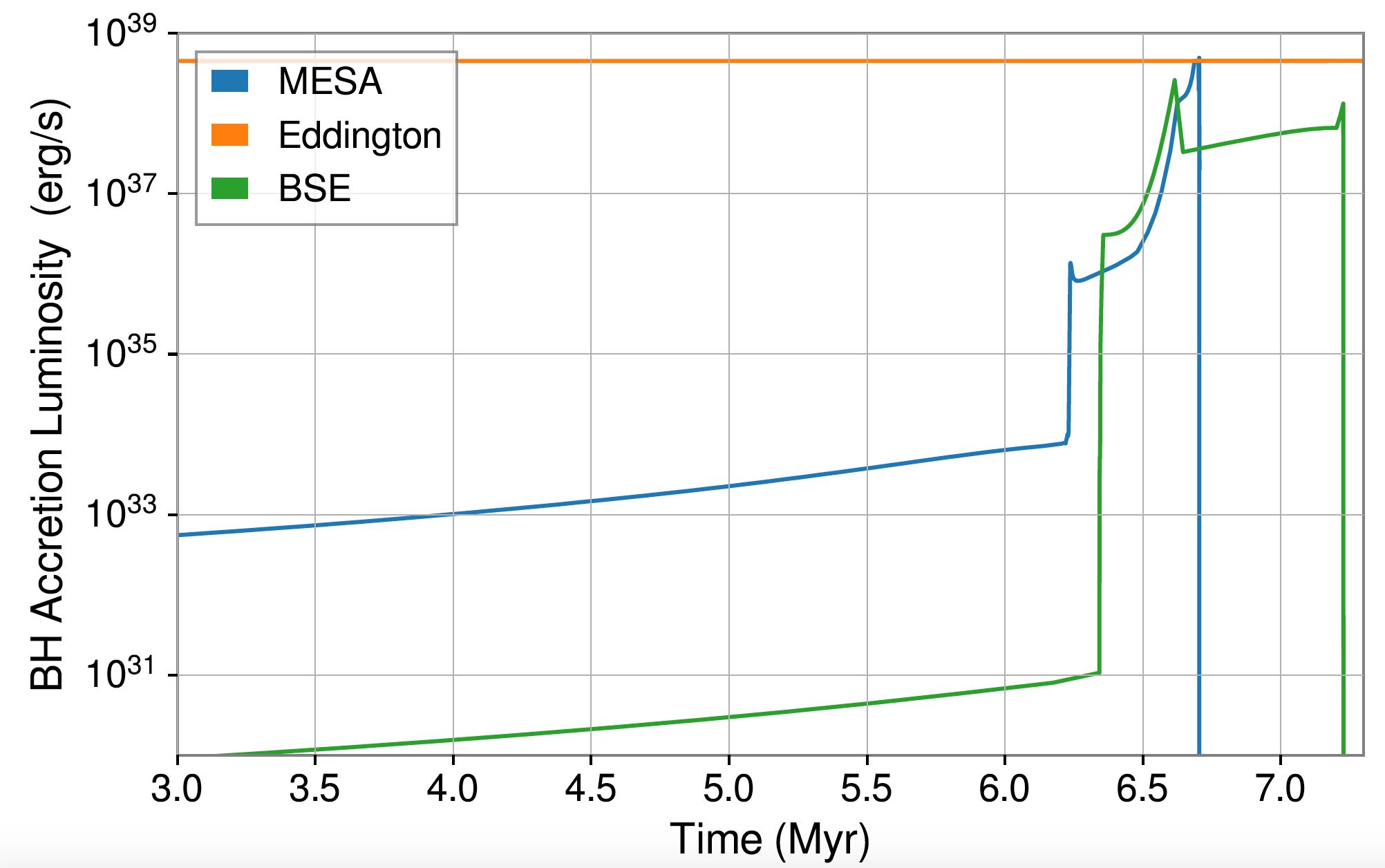
In these individual simulations, we evolve systems beginning in the HMXB phase, whereas in COSMIC we evolve binaries initialized with two randomly sampled main sequence stars. In the above plot, both MESA and BSE simulations begin with an initial star mass of 31.6 solar masses, an initial black hole mass of 3.2 solar masses, an initial orbital period of 1950 days, an initial eccentricity of zero, and a metallicity of one-tenth solar metallicity. In addition to the MESA and BSE luminosity tracks, we plot the Eddington luminosity as an upper limit for the emission.
From this plot, we see that MESA and BSE exhibit comparable mass transfer behavior leading up to peak emission. However, after peak emission, the MESA luminosity immediately drops to zero while the BSE luminosity remains observable for an additional 0.5 Myr. We believe that this is a result of differing wind mass transfer prescriptions in MESA and BSE, but more rigorous testing of these features is required to fully understand what is going on.
Future Steps: This project is set to continue through May 2020. As mentioned previously, the next project step is to determine how long our simulated binaries emit at observable luminosities. This will allow us to calculate the probability that we can see them in real life. In addition, a more detailed analysis of the COSMIC and MESA simulations is required to understand the finer details in our results, as discussed above.